Research Impressions
The research focus at the Chair for Continuum Biomechanics and Mechanobiology can be classified into Modelling the Musculoskeletal System, which is predominantly driven by Computational and Experimental Research Questions, and selected Biomechanical Application-driven Research Questions.
qMOTION - Simulation-enhanced Highdensity Magneto-myographic Quantum Sensor Systems for Decoding Neuromuscular Control During Motion (ERC-AdG 2021)
Being able to decode neural signals that control skeletal muscles with high accuracy will enable scientific breakthroughs in diagnostics and treatment, including early detection of neurodegenerative diseases, optimising personalised treatment or gene therapy, and assistive technologies like neuroprostheses. This breakthrough will require technology that is able to record signals from skeletal muscles in sufficient detail to allow the morpho-functional state of the neuromuscular system to be extracted. No existing technology can do this. Measuring the magnetic field induced by the flow of electrical charges in skeletal muscles, known as Magnetomyography (MMG), is expected to be the game-changing technology because magnetic fields are not attenuated by biological tissue. However, the extremely small magnetic fields involved require extremely sensitive magnetometers. The only promising option is novel quantum sensors, such as optically pumped magnetometers (OPMs), because they are small, modular, and can operate outside of specialised rooms. Our vision is to use this technology and our expertise in computational neuromechanics to decode, for the first time, neuromuscular control of skeletal muscles based on in vivo, high-density MMG data. For this purpose, we will design the first high-density MMG prototypes with up to 96 OPMs and develop custom calibration techniques. We will record magnetic fields induced by contracting skeletal muscles at the highest resolution ever measured. Such data, combined with the advanced computational musculoskeletal system models, will allow us to derive robust and reliable source localisation and separation algorithms. This will provide us with unique input for subject-specific neuromuscular models. We will demonstrate the superiority of the data over existing techniques with two applications; signs of ageing and neuromuscular disorders and show that it is possible to transfer these methodologies to clinical applications.
Modelling the Musculoskeletal System
Mathematical models and computer simulations have the great potential to substantially increase our understanding of the biophysical behaviour of the neuromuscular system. This requires detailed multiscale and multiphysics models, which, once validated, allow systematic in silico investigations that are not necessarily feasible within experiments. The aim of our group is the development of a detailed biophysical model of the entire neuromuscular system. We consider the neuromuscular system as an integrated physiological system and thus, we combine models of motor neuron pools, the sensory system and multiscale models describing the mechanical behavior of skeletal muscles. By employing sub-models that are based on strictly biophysical modeling approaches, the resulting model closely represents the underlying physiological system and thus has the potential to provide valuable insights into the complex interrelations within the healthy system and pathological conditions.
Active research focus areas:
- Analyzing the influence of sensory signals on the output of motor neuron pools.
- Developing detailed biophysical models of muscle spindles.
- Investigating the impact of the three-dimensional motor unit distribution within skeletal muscles on the force output, strain and pressure distribution.
Review on the neuromuscular system:
Röhrle O, Yavuz US, Klotz T, Negro F, Heidlauf T. Multiscale modeling of the neuromuscular system: Coupling neurophysiology and skeletal muscle mechanics. Wiley Interdisciplinary Reviews: Systems Biology and Medicine. 2019
Skeletal muscles can be voluntarily controlled by the somatic nervous system and enable motion and active force production. From a physiological point of view, the smallest functional unit of the neuromuscular system is the motor unit. A Motor unit consists of a motor neuron and all the muscle fibers it innervates. The fact that a muscle consists of many (up to several hundreds) of motor units allows to finely modulate the force output and thus enables complex motion. Specifically considering the biophysical function of skeletal muscles, the active contractile behavior of the tissue is dictated by the microstructure of the tissue and the cellular working principles of the muscle fibers. For example, after stimulating the muscle fiber membrane with an action potential, calcium release from the sarcoplasmic reticulum into the cell is triggered. Serving as a second messenger calcium enables enables cross-bridge cycling, i.e. controlling skeletal muscles molecular motor.
Our chemo-electro-mechanical skeletal muscle models resolve all key aspects of skeletal muscles anatomy and physiology within an organ-scale continuum model. In detail, by employing a multi-scale approach the model predicts the electrical potential fields within the tissue, the biochemical behavior of the muscle cells and the deformations of the tissue induced by either physiological or other external inputs.
Thus, the chemo-electro-mechanical skeletal muscle model is e.g. applied to
- systematically analyze the influence of individual structures or phenomena on the whole system,
- generate virtual EMG signals, which are for example useful to evaluate the goodness of automatic decomposition algorithms,
- predict the outcome of external electrical stimulation protocols.
We further aim to improve the physiological accuracy of the model by
- incorporating additional structures and phenomena such as muscle spindles or the metabolic behavior of the muscle cells,
- developing new homogenization methods, which are essential for consistent and efficient multi-scale models.
Since the chemo-electro-mechanical skeletal muscle model is computationally expensive we also constantly optimize the efficiency of the simulations by
- develop or apply suitable numerical solution methods,
- employ problem specific parallelization strategies.
Modelling skeletal muscle tissue within the framework of continuum mechanics demands suitable constitutive material models on the macroscopic (organ) scale. This is also the fundamental requirement for reliable three-dimensional muscle simulations using the finite-element method. Commonly used phenomenological constitutive approaches, which have to be calibrated to macroscopic experimental material tests, suffer from the high inter- and intra-tissue variability of skeletal muscle tissue and the lack of individual experiments for the specific type of muscle to be modelled. The high variations in the tissue properties are hereby originated in the highly heterogeneous microstructure of the tissue.
Hence, our research group focuses on the development and consistent formulation of novel multi-scale material models for skeletal muscle tissue. These models directly take into account the significant microstructural features, for which a lot of experimental data is available. For instance, the model directly incorporates a comprehensive formulation for the collagenous structures of the extracellular matrix, including the description of collagen fibre stiffness and the dispersion of collagen fibres. Suitable homogenisation methods are developed and applied to get the wanted macroscopic constitutive behaviour. In contrast to phenomenological approaches, the proposed multi-scale model and its underlying idea of a rigorous bottom-up framework is able to answer questions like, for instance:
- How do microstructural changes (for example, due to diseases) influence the overall behaviour of skeletal muscles?
- What kind of direction-dependent behaviour on the organ scale results from the arrangement of structures on the microscale?
- How does the stiffness of single collagen fibres influence the stress distribution of the whole muscle?
The novel multi-scale model is an important step towards subject-specific material models.
This is done in close collaboration with the University of Pennsylvania, USA.
Skeletal muscles play an important role in overall health; from movement range, resistance and strength, to protein reservoir, muscles have the ability to adapt to any specific intensity of physical activity. A particular adaptation feature can be targeted by means of exercise. In principle, there are two kinds of training protocols: training for resistance and training for endurance, but a combination of these two protocols lead to intermediate outcomes.
Exercise, mechanical, or electrical stimuli trigger biochemical responses known as signaling pathways. These pathways control the adaptation processes at the cell level. Muscle cells are also known as muscle fibers, and there are many fiber types that share characteristics with one of two categories: some muscle cells (known as Type I fibers) adapt to sustained activities such as walking, or running a marathon, and are fatigue resistant; other cells (known as Type II fibers) adapt to activities that require force or power, such as sprinting, but are easily fatigable. As any muscle has a different proportion of fiber types, an adaptation process involves the transition between fiber types.
The aim of this research is to model the hypertrophy, atrophy, and fiber shifting processes of muscle tissue as the outcome of different training protocols. We assume that the training input stimulates a biochemical system that drives the protein content and fiber shifting of the tissue, and as a consequence, the model predicts force and fatigue adaptation. This is in collaboration with the National University of Colombia (Bogotá).
Real-world human movement generally arises from an interaction of multiple muscles. Experimental techniques may be used to measure outputs of the human movement system, e.g., via electromyography, sonomyography and/or optical techniques and while this data may be used to infer the underlying mechanisms behind human movement, e.g., using decomposition techniques to predict motor-neuron firings for a particular movement, there still remains a very large amount of uncertainty since the exact inputs to the system are not known.
Therefore, our research focuses on the use of physiologically realistic multi-muscle simulation models to better understand the underlying mechanisms of healthy and pathological human movement.
Active research focus areas:
- Upper arm modelling, consisting of six muscles capable of flexion/extension and supination/pronation to investigate muscle and motor-unit synergies for applications in ergonomics, human-machine -interaction and sports and rehabilitation
- Pervasive simulation to enable resource-poor systems like laptops or tablets to perform complex simulation tasks. For example by using cloud computing to distribute the work-load, or machine learning methods to benefit from a (offline) learning processes.
- Surrogate modelling techniques to create a computationally highly efficient version of a model of the human upper arm for real-time simulation and visualization and interaction in virtual and/or augmented reality.
- Use of surrogate models in our investigations of different activation strategies to generate desired movements and possibly even feedback mechanisms.
This is in close collaboration with the IPVS and VISUS at the University of Stuttgart and the Fraunhofer IPA.
Computationally Driven Research
Modelling the musculoskeletal system using a continuum-mechanical approach based on the theory of finite hyperelasticity, together with fine finite element discretisations is not only a challenging, but also a computationally expensive task. Especially in the many query context, which for example can arise when patient specific data is needed, or if parameter studies for healthy and pathological conditions shall be conducted, the simulations become prohibitively expensive or even unfeasible. For this reason, one needs to find ways to reduce the computational costs and this is where the mathematical field of model order reduction (MOR) suggests itself.
One possibility of reducing the computational effort of simulating a high dimensional system of differential equations, in the context of MOR referred to as full order model (FOM), is the projection based MOR. Essentially, this approach aims at reducing the number of degrees of freedom of the system by projecting it onto a lower dimensional subspace of the original high dimensional solution space. That way, a reduced order model (ROM) of lower dimension is obtained. For the determination of a suitable low dimensional subspace, the proper orthogonal decomposition (POD) is a well established and widely applied method. The idea of the POD is based on a split of necessary computations into an offline and an online phase. Therein, it is assumed, that during the offline phase, time and resources are unlimited, i.e. computations involving high dimensional operations can be executed, while aiming at computing only operations of low dimensional complexity during the online phase.
The challenge of constructing a fast and stable skeletal muscle model lies in considering and preserving the structural properties of the FOM when trying to set up a suitable method to obtain the ROM. Properties we focus on are
- dynamics
- incompressibility
- nonlinearity
which means we obtain a nonlinear differential algebraic equation system (DAE) with three fields to be solved for and a saddle point problem in the linear solve.
A summary of the research activities within this field will be published soon.
A summary of the research activities within this field will be published soon.
Experimentally Driven Research
Biofeedback, Rehabilitation therapies as well as the adaption of active prostheses and orthoses demand a sophisticated interpretation of muscle signals. Thereby, the attachment of sensors, i.e. electrodes, comes along dealing with different impacts. Our aim is to improve the understanding of muscular activity, its conditional behavior and the source of its generation, using high-sensity electromyography (EMG). Moreover, the whole functional scope is going to be addressed by the investigation: from surface expressions to the characteristics in the central nervous system.
The EMG is a diagnostic technique to record electrical potentials from the skeletal muscle. The electromyographic signal consists of motor unit action potentials innervating the muscle fibers contributing to a comprehensive contraction using specific strategies in motor control, depending on multiple factors, as load and intention, for example. The decomposition of the signal reveals the contribution of different neural influences, like afferent sensory input, spinal and cortical shares eventually leading to a movement. Proper acquisition in experiments and reliable processing are the fundamentals of this analysis.
In biomechanical modeling of muscles, knowledge on the mechanical properties of the muscle, such as the geometry, is necessary. One widely used technique with a short acquisition time is ultrasonic imaging of the muscles. Most often, muscles are imaged with ultrasound in a two-dimensional view. There are also concepts existing for three-dimensional ultrasound measurement of muscle tissue in order to acquire the whole muscle geometry. Here, the transducer moves along the muscle’s longitudinal axis obtaining several transversal images which can be reconstructed to a 3D image. Although these methods show reasonable results, they are subject to various limitations such as the need of an expensive optical motion capture system in order to know the translation and rotation between two images.
Therefore, in this project a rig which holds the ultrasound transducer and automatically moves it to scan one whole muscle will be developed. Using encoders to track positions enables 3D ultrasound imaging without the necessity of an expensive motion capture system and other settings bound to a lab environment.
More information willl be published here soon.
This research is funded by the newly established Terra Incognita Fund of the University of Stuttgart.
Selected Biomechanical Applications
Percutaneous vertebroplasty is a procedure in which liquid polymethylmethacrylate (PMMA), i.e. bone cement is injected into a structurally weakened vertebral body. The bone cement undergoes a curing process which then stabilises the vertebral column. However, the cement infiltration behaviour is often hard to predict since it is affected by many biomechanical factors, which in some cases can lead to cement leakage and subsequent complications. A multi-constituent material-injection-process model could significantly add value for improving the procedure of vertebroplasty by preventing such cases. Since the inside of a vertebral bone is porous, the injection process can be modelled as porous media flow using the well-known Theory of Porous Media (TPM) via homogenisation of the constitutive phases.
The main challenges of the project are:
- To develop a macro-scale TPM model that can be used as a tool to determine operating parameters specific to individual patients undergoing vertebroplasty
- To study the pore-scale processes and homogenise them in such a way so as to improve and effectively use the macro-scale model
- To design and exploit experimental set-ups for validating the computational models
This project is funded by the SFB1313 and is in close collaboration with the AO Research Institute at Davos.
Partial and/or complete tooth loss strongly influences quality of life and its prevalence is likely to increase given an aging word-wide population. Undesired mechanical loading is a major factor in dental implant failure, which may arise due to improper implant selection or design and/or improper osseointegration. As such, a better understanding of the local deformation and loading of dental implants and surrounding dental structures may lead to better and more robust dental implant designs.
Our research aims to build a virtual masticator which realistically predicts the local deformations within the dental structures during natural chewing, biting and clenching motions. The use of physiologically realistic boundary conditions leads to more realistic predictions of the local deformations and loading of the teeth/implants and surrounding dental structures and ultimately leads to a better understanding of dental implant design and dental pathologies.
Research focus areas are:
- Developping novel methods to determine patient specific, in-vivo, biting forces and occlusal load maps
- Assist dental implant design under realistic occlusal loads
- In collaboration with Fraunhofer IPA (Biomechatronic Systems), the use of a chewing robot with a food repositioning system capable of simulating mastication for validation and food-stuff development
More information about this project will be published soon.
The present work focuses on investigating the cause-effect factors that link the destructive remodelling processes of an aortic valve's (AV) extracellular matrix (ECM) with ventricular diseases. To achieve that goal, 3-D finite element models (FEM) of the human AV are constructed based on the assumption that the function, deformation and performance of the valve are strongly dependent on its geometry. The initial valvular geometries employed for the models are obtained from non-invasive medical imaging data like MRI, 3D-TEE and CT; and by user-(semi)independent feature extraction and image processing methods with the aim of replicating the particularities of the patient- and case-specific scenarios.
Moreover, the construction of the above mentioned models is rounded by the implementation of suitable constitutive models describing the valvular tissue's behavior, which are derived from experimental data as well as from literature. Case-specific boundary conditions are also applied to the models to reproduce the bending and tensile stretches suffered by the cusps and caused by the changing ventricular and aortic pressure patterns along the heart cycle. These pressure loads are assumed to be ranging from typical pressures occurring in healthy left ventricles to pressures that occur in left ventricles exhibiting different stages and types of cardiomyopathy.
With the integration of all the above mentioned features into the FEM along with a physiologically accurate tissue's fibre orientation, it is possible to generate an insight of the dynamics and the stress-stretch distribution of the main valve components at relevant stages of the valves opening and closing functions. As a result, valuable data is generated that allows us to compare the stress and deformation patterns computed with the models with the calcification patterns incidence and progression as well as with the dynamics of the patients' valves observed in medical imaging data. The latter, is the chosen path to study the development of ECM ruptures and remodelling, which potentially develops into calcific aortic valve disease from an initial to end-stage calcification along the valvular tissue.
Vera Tahedl
Administrative Associate (Röhrle group)
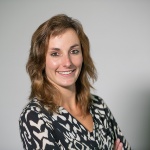
Sina Schorndorfer
Coordinator GRK 2198 / SPP2311